Virus-like particles expressing microneme-associated antigen of Plasmodium berghei confer better protection than those expressing apical membrane antigen 1
Article information
Abstract
Malaria is a global disease affecting a large portion of the world’s population. Although vaccines have recently become available, their efficacies are suboptimal. We generated virus-like particles (VLPs) that expressed either apical membrane antigen 1 (AMA1) or microneme-associated antigen (MIC) of Plasmodium berghei and compared their efficacy in BALB/c mice. We found that immune sera acquired from AMA1 VLP- or MIC VLP-immunized mice specifically interacted with the antigen of choice and the whole P. berghei lysate antigen, indicating that the antibodies were highly parasite-specific. Both VLP vaccines significantly enhanced germinal center B cell frequencies in the inguinal lymph nodes of mice compared with the control, but only the mice that received MIC VLPs showed significantly enhanced CD4+ T cell responses in the blood following P. berghei challenge infection. AMA1 and MIC VLPs significantly suppressed TNF-α and interleukin-10 production but had a negligible effect on interferon-γ. Both VLPs prevented excessive parasitemia buildup in immunized mice, although parasite burden reduction induced by MIC VLPs was slightly more effective than that induced by AMA1. Both VLPs were equally effective at preventing body weight loss. Our findings demonstrated that the MIC VLP was an effective inducer of protection against murine experimental malaria and should be the focus of further development.
Introduction
Malaria, caused by several members belonging to the genus Plasmodium, is an endemic disease affecting millions of individuals residing in tropical and subtropical regions. In 2021, approximately 247 million individuals were diagnosed with malaria, with 600,000 associated deaths [1]. Fortunately, global efforts to eradicate malaria have contributed to reducing malaria transmission and malaria-related deaths in many endemic countries [2]. This is mainly attributed to chemotherapeutic intervention, as evidenced by the 95% treatment efficacy of artemisinin combination therapy against P. falciparum malaria [3]. However, drug resistance and artemisinin combination therapy treatment failures have been reported on 3 continents [4–6]. Thus, alternative approaches that impede the emergence of drug-resistant Plasmodium spp. are needed.
A potential solution to delay or even reduces the probability of resistance evolution is to lower the overall number of malaria cases through vaccination. Resistance to vaccines is far less likely to evolve than that to drugs, and even if it does develop, it is less likely to impact the well-being of hosts [7]. Consequently, vaccines are currently being used to address antimicrobial resistance because they significantly lower the emergence of escape mutants [8]. Furthermore, vaccination is one of the most cost-effective methods for controlling infectious diseases. Malaria is no exception, and a mathematical modeling study revealed that the introduction of antimalarial vaccines could be a major factor benefiting public health in sub-Saharan Africa [9]. RTS,S/AS01 and R21/Matrix-M are adjuvant VLP vaccines that have been approved for use by the Food and Drug Administrations of several African countries, as well as the WHO [10]. Unfortunately, phase 3 clinical trial results for the first malaria vaccine (RTS,S/AS01) revealed failure to induce long-lasting immunity [11]. This led to the development of the R21 vaccine formulated with the saponin-based Matrix-M adjuvant, which provided a more durable immune response than RTS,S/AS01. The safety and efficacy of the R21/Matrix-M vaccine were evaluated in a recent phase 3 clinical trial involving more than 4,800 African children, revealing an overall efficacy of 68–75% depending on the location and presence of seasonal variations, but this waned over time [12]. Thus, additional efforts for vaccine development are necessary.
Owing to its highly immunogenic nature, paired with the endorsement of the aforementioned VLP vaccines for use in malaria-endemic regions, many experimental malaria vaccine studies involving this platform have been undertaken. For example, self-assembled norovirus-based nanoparticles expressing either the circumsporozoite protein or the cell-traversal protein for ookinetes and sporozoites of P. falciparum conferred protection in mice [13]. Another study conjugated the ookinete surface antigen PSOP25 to the AP205 bacteriophage protein for VLP assembly, and this vaccine conferred moderate transmission-blocking activity [14]. Although most studies have focused on developing transmission-blocking vaccines [15,16], some have attempted to develop an effective blood-stage vaccine [17,18]. We previously developed blood-stage VLP vaccines expressing apical membrane antigen 1 (AMA1), either alone or in combination with microneme-associated antigen (MIC), and their efficacies were evaluated following P. berghei challenge infection in mice [19,20]. However, a comparative study on the vaccine efficacy of these 2 antigens has not been conducted. To address the limitations of our previous work, this study compared the protective efficacy elicited by VLPs expressing either AMA1 or MIC to identify which was more suitable for further development as a malaria vaccine candidate antigen.
Materials and Methods
Ethics statement
All experimental protocols were approved by the Kyung Hee University IACUC (KHUIBC (SE)-19-034), and all animal experiments were conducted according to the IACUC guidelines. Immunization and blood collection were performed under mild anesthesia induced and maintained with isoflurane. All efforts were made to minimize the number of experimental animals used and their suffering.
Animals, parasites, cells, and antibodies
Seven-week-old female BALB/c mice were purchased from NARA Biotech (Seoul, Korea). P. berghei strain ANKA was maintained in BALB/c mice and used for challenge infection as described previously [20]. Trichinella spiralis was maintained in Sprague Dawley rats as described previously [21]. Spodoptera frugiperda insect cells (Sf9) were cultured in serum-free SF900 II media (Invitrogen, Carlsbad, CA, USA) to produce recombinant baculovirus and VLPs. Sera from P. berghei-infected mice were collected and used to detect P. berghei AMA1 and MIC proteins in VLPs by ELISA and western blotting. Horseradish peroxidase (HRP)-conjugated goat anti-mouse IgG was purchased from SouthernBiotech (Birmingham, AL, USA). Sera collected from unimmunized P. berghei were used as infection controls for western blotting.
P. berghei and T. spiralis excretory-secretory (ES) antigen preparation
P. berghei strain ANKA was used as a coating antigen for ELISA and for fluorescence-activated cell sorting and was prepared as described previously [20]. Briefly, RBCs from P. berghei-infected mice were collected by centrifugation at 240 g for 10 min at 4°C and incubated with 0.15% saponin prepared in phosphate-buffered saline (PBS) for 10 min at 37°C. P. berghei parasites released from the RBCs were washed, centrifuged at 1,320 g for 1 min at 4°C, and sonicated. The lysates were stored at −20°C until use.
The preparation of T. spiralis as a coating antigen for ELISA was performed as described previously [21]. After digesting the muscle tissues of T. spiralis-infected rats in pepsin-HCl solution at 37°C, the contents were repeatedly washed, and larvae present in the muscle were isolated under microscopy. ES antigens of T. spiralis were acquired by culturing in incomplete DMEM (Welgene, Gyeongsan, Korea) at 37°C with 5% CO2 for 3 days. The medium containing the ES antigens was collected by centrifugation at 3,000 rpm for 10 min at 4°C and stored at −20°C until use.
Generation of AMA1 and MIC VLPs
P. berghei VLPs expressing either AMA1 or MIC were prepared and characterized as described previously [19]. In brief, the complete gene sequences of P. berghei AMA1 (GenBank: XM_672965.2) and MIC (GenBank: XM_034568180) underwent codon optimization and were cloned into pFastBac vectors, and clonal constructs expressing these codon-optimized genes were purchased from GenScript (Piscataway, NJ, USA). The clones were transformed into competent DH10Bac cells, and bacmid DNA was acquired from the transformants. Sf9 cells were transfected with the bacmid DNA for AMA1 and MIC recombinant baculovirus assembly.
Confirmation of gene insertion and VLP characterization
The codon-optimized vectors containing the 2 genes of interest were subjected to restriction enzyme digestion with EcoRI and HindIII. DNA bands were visualized via gel electrophoresis, and their sizes were validated using a size marker. For western blotting, VLPs were resolved on a 10% SDS-PAGE gel and transferred to a nitrocellulose membrane. After blocking the membranes with 5% skim milk prepared in Tris-buffered saline with Tween-20, they were probed overnight at 4°C with polyclonal sera acquired from P. berghei-infected mice. The next day, the membranes were incubated with HRP-conjugated anti-mouse IgG for 1 h at room temperature. Bands were developed using enhanced chemiluminescence and visualized using a ChemiDoc imaging system (Bio-Rad, Hercules, CA, USA). The VLPs were also visualized using transmission electron microscopy (TEM).
Immunization and challenge infection
BALB/c mice (n=10) were immunized intramuscularly 3 times at 4-week intervals with 100 μg of either AMA1 or MIC VLP. Then, they were intraperitoneally challenged with 0.5×104 P. berghei parasites resuspended in 100 μl PBS as described previously [20]. At 6 days postinfection (dpi), 5 mice in each group were sacrificed to collect blood, the spleen, and inguinal lymph nodes (ILNs). The remaining mice were monitored for changes in parasitemia and weight loss.
Antibody responses in sera
Sera from mice were collected using retro-orbital plexus puncture 3 weeks after each immunization, and naïve serum was used as the negative control. Antibody responses to P. berghei antigen, AMA1 VLPs, and MIC VLPs were determined by ELISA as previously described [19]. Briefly, 0.5 μg/ml of either of the 2 VLPs or 2 μg/ml of P. berghei whole lysates dissolved in carbonate coating buffer were used as coating antigens. The coated wells were blocked with 0.2% gelatin, and serially diluted sera were inoculated into the respective wells. Following 1 h of incubation at 37°C, HRP-conjugated anti-mouse IgG was added, and the plates were incubated at 37°C for 1 h. Substrate buffer containing o-phenylenediamine (Sigma-Aldrich, St. Louis, MO, USA) and H2O2 was added to each well for color development. Reactions were halted with diluted H2SO4, and the optical density was measured at 492 nm.
Flow cytometry analysis of immune cell responses
Flow cytometry was performed to determine the levels of CD4+ T cells and germinal center (GC) B cells in the ILN and blood of mice. After stimulating the cells with 0.5 μg/ml of sonicated P. berghei whole lysate antigen, the Fc receptors were blocked using FcBlock antibody (clone 2.4G2; BD Biosciences, San Jose, CA, USA). Cells were stained using fluorophore-conjugated antibodies (CD3e-PE-Cy5, CD4-FITC, B220-FITC, and GL-7-PE; BD Biosciences) and sorted using an Accuri C6 Flow Cytometer (BD Biosciences). A total of 10,000 events were acquired, and the data were analyzed using C6 software (BD Biosciences).
Cytokine responses in mice spleens
Spleens were collected at 6 dpi and processed as described previously [22]. Briefly, individually processed spleen homogenates were centrifuged at 2,000 rpm for 10 min, and the supernatants were collected for cytokine analysis using OptEIA tumor necrosis factor-α (TNF-α), interferon-γ (IFN-γ), and interleukin-10 (IL-10) ELISA kits (BD Biosciences) according to the manufacturer’s instructions.
Parasitemia burden assessment
Blood samples that were collected from challenge-infected mice using retro-orbital plexus puncture every 3–5 days were used to assess parasitemia as previously described [20]. In brief, the blood samples were collected in microcentrifuge tubes containing PBS and 500 U/ml heparin. After staining the RBCs with 1 μl SYBR Green I (Invitrogen) and incubating for 30 min, parasitemia was determined by flow cytometry analysis.
Statistical analysis
All parameters were recorded for individuals within all groups. All data were presented as mean±SD, and statistical significance between groups was analyzed by one-way analysis of variance using GraphPad Prism v6.0 (GraphPad, San Diego, CA, USA).
Results
Characterization of the 2 VLPs expressing P. berghei antigens
Codon-optimized P. berghei AMA1 or MIC gene insertion into pFastBac vectors was confirmed following restriction enzyme digestion with EcoRI and HindIII and the subsequent detection of DNA bands of approximately 1.5 kb and 1 kb, respectively. AMA1 and MIC protein expression on the VLPs was confirmed by western blotting. When probed with polyclonal anti-P. berghei antibody, bands corresponding to AMA1 and MIC proteins were observed at 60 and 35 kDa, respectively. To confirm the parasite specificity of anti-P. berghei polyclonal antibody, ELISA was performed using serially diluted antigens, and T. spiralis ES antigens were used as a negative control. Anti-P. berghei polyclonal antibody did not react with T. spiralis antigens regardless of the coating antigen concentration. However, the sera specifically detected AMA1 and MIC antigens in a dose-dependent manner. At 62.5 ng/well, sera were incapable of specifically detecting parasitic antigens. Proper assembly of the VLPs was confirmed by TEM analysis. Both AMA1 and MIC antigens were self-assembled in Sf9 cells along with the influenza M1 antigen, which served as a docking site for these antigens. All of the characterization data are provided in the Supplementary Fig. S1.
Antigen- and parasite-specific antibody detection
Mice were immunized and experiments were conducted as scheduled (Fig. 1A). Vaccine-induced antibody responses were determined by ELISA using sera collected at regular intervals. AMA1-specific IgG levels were detected as early as week 3 after the priming immunization, but the antibody responses waned substantially at a 900-fold dilution. In contrast, strong AMA1-specific responses were maintained following 2 consecutive booster immunizations, even at a 2,700-fold dilution (Fig. 1B). MIC VLP immune sera also demonstrated antigen specificity. Priming mice with MIC VLPs did not elicit a substantial increase in MIC-specific antibody responses. The first booster immunization enhanced the MIC-specific antibody response to some extent, but this reached priming serum levels at a 900-fold dilution. After the final immunization, MIC-specific IgG responses were maintained throughout, even at an 8,100-fold dilution (Fig. 1C). To ensure that these antigen-specific responses interacted with P. berghei parasites, ELISA was performed using P. berghei whole lysate antigens (Fig. 1D). For both AMA1 and MIC VLPs, the number of vaccinations was positively correlated with parasite-specific serum IgG levels.
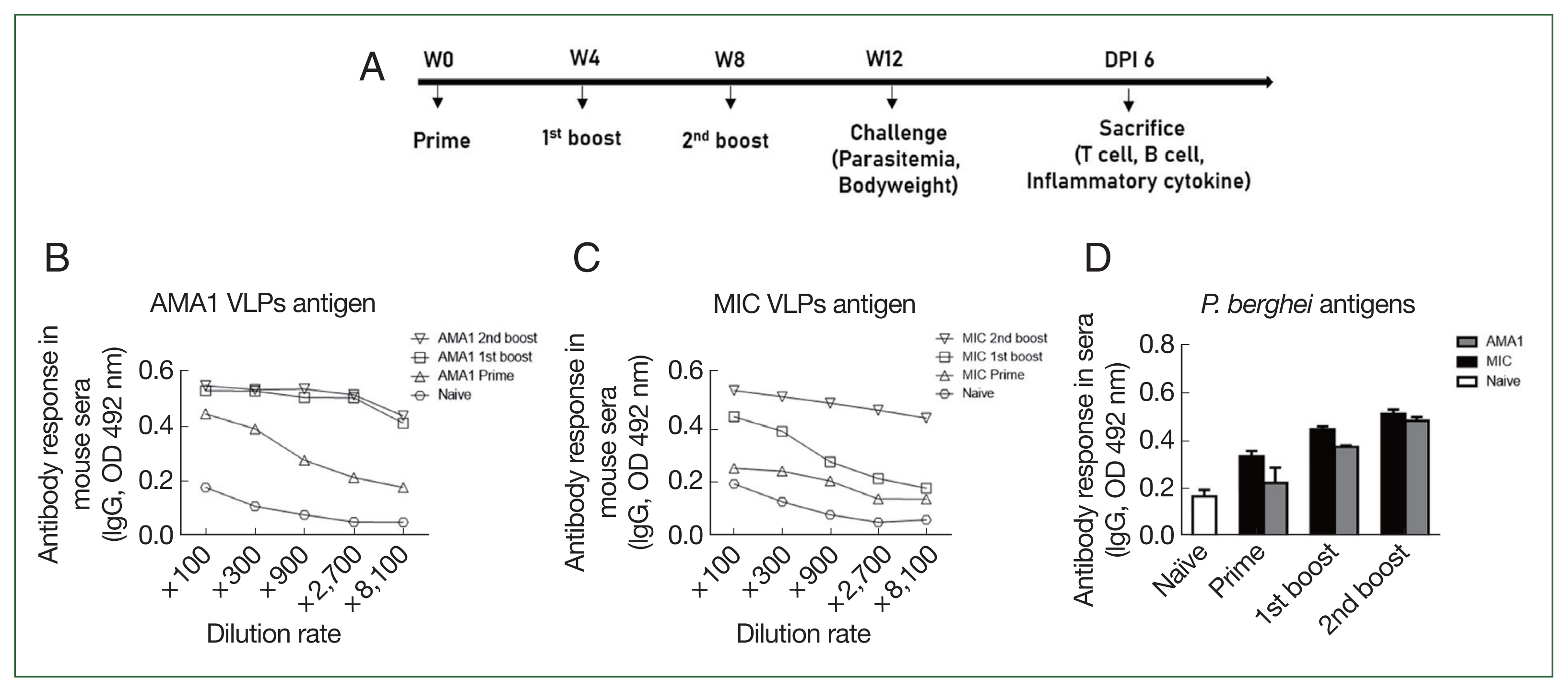
Immunization scheduling and vaccine-induced antibody response detection. Schematic depicting the animal experimental schedule (A). Antigen specificity of AMA1 and MIC antibodies evaluated by ELISA in sera of VLP-immunized mice collected at regular intervals (B, C). Parasite specificity of the 2 immune sera tested against the whole lysate antigen of P. berghei (D). Data are presented as mean±SD.
VLP vaccines elicit cellular immunity and contribute to protection
Blood and ILN cells were collected at 6 dpi, and single-cell populations from both samples were acquired using flow cytometry. Both AMA1 and MIC VLPs enhanced the frequencies of GC B cells in the ILNs of mice compared with the unimmunized controls (Fig. 2A and B). Interestingly, MIC VLPs induced significantly greater GC B cell frequencies than AMA1 VLPs. In contrast to the GC B cell responses, there were no drastic changes in blood CD4+ T cell frequencies. Of the 2 VLPs, only the MIC VLP immunization elicited significantly enhanced CD4+ T cell induction, whereas AMA1 VLPs did not (Fig. 2C).
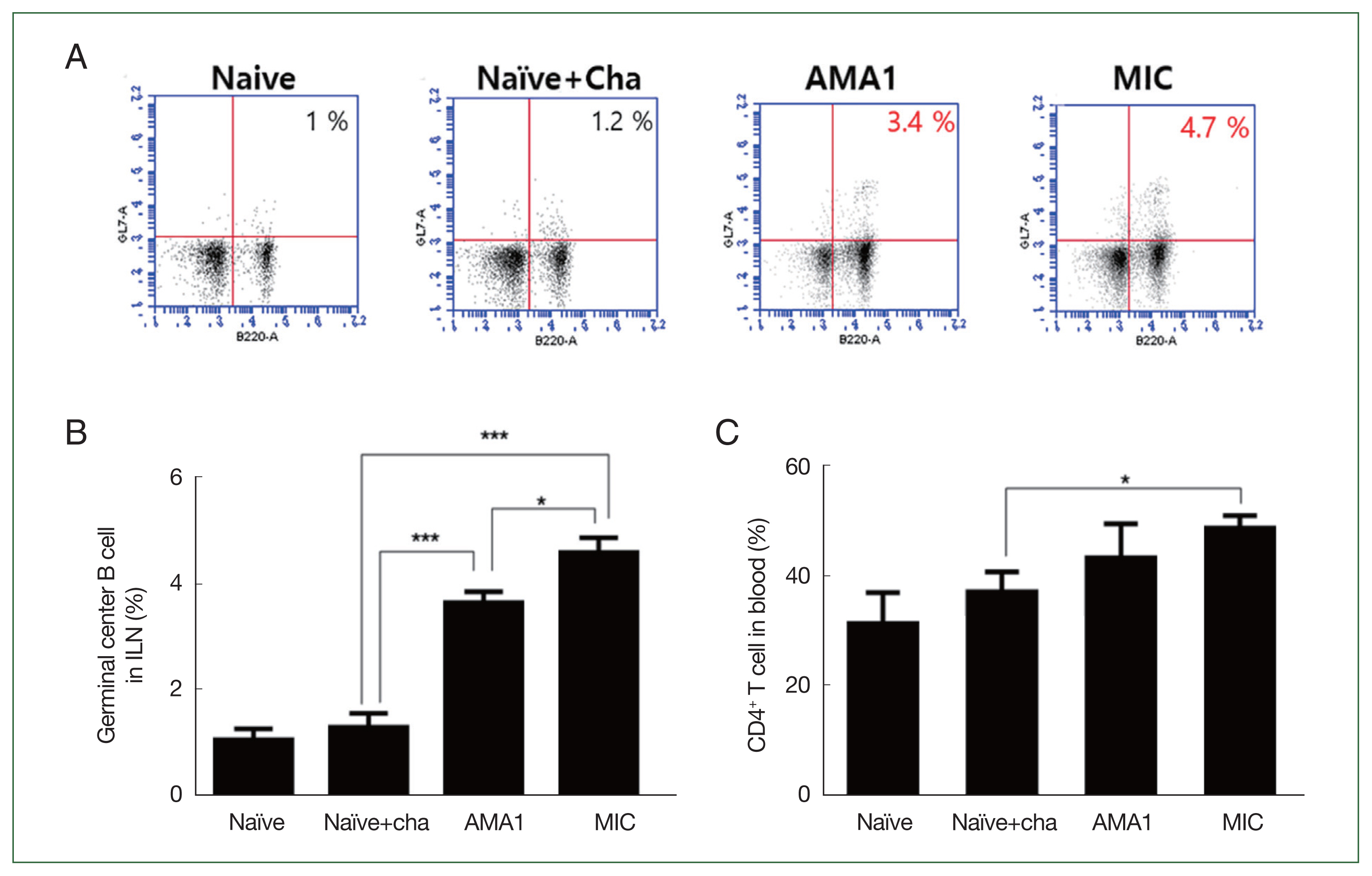
Flow cytometry analysis of GC B and CD4+ T cells. Single-cell suspensions were acquired from ILN and blood for GC B cell and CD4+ T cell quantification, respectively. Frequencies of GC B cells (A, B) and CD4+ T cells (C) assessed using flow cytometry. Data are presented as mean±SD. *P<0.05 and ***P<0.001.
VLP vaccines suppress the cytokine profiles of immunized mice
Splenic cytokine production was measured in P. berghei-infected mice using ELISA. Compared with the naïve+challenge infection controls, mice immunized with either AMA1 or MIC VLPs produced significantly less TNF-α, and the level was comparable with that of the naïve control (Fig. 3A). In contrast, profound reductions in the inflammatory cytokine IFN-γ were not detected in either vaccination groups (Fig. 3B). Despite the immunizations, IFN-γ levels for MIC VLPs were similar to those of the naïve+challenge control. Reduced IFN-γ was detected for AMA1 but compared with the control group, the changes were not statistically significant. Similar to TNF-α, mice immunized with either of the 2 VLPs showed significantly reduced IL-10 production (Fig. 3C). There were no statistically significant differences between the 2 immunization groups for any of the 3 cytokines.
Parasitemia and body weight changes in immunized mice
VLP vaccine efficacy was determined by parasitemia assessment of blood samples collected at regular intervals from immunized mice infected with P. berghei. Compared with the unimmunized controls, differences in parasitemia were initially negligible, but gradual changes became noticeable from 22 dpi onward. Significant differences in parasitemia were observed in the vaccination groups after 35 dpi (Fig. 4A). At 45 dpi, parasitemia was reduced by 1.5- and 2-fold in the AMA1 and MIC VLP groups, respectively (Fig. 4B).
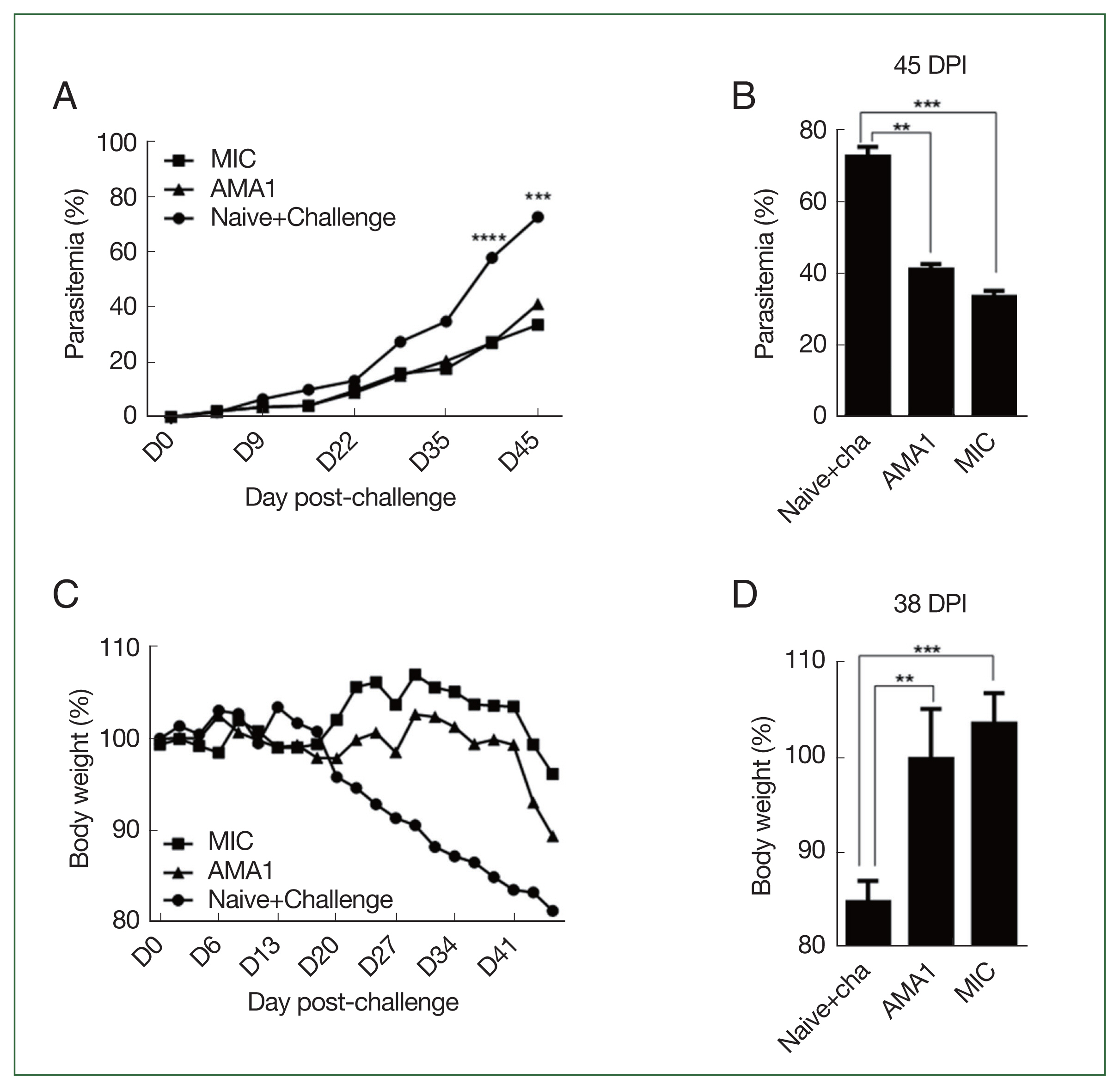
Parasite burden and malaria-induced weight loss. Changes in parasitemia and body weight of mice were recorded at regular intervals. Parasitemia in the blood of P. berghei-infected mice was monitored over 45 days (A), and changes were compared at 45 dpi (B). Weight changes of P. berghei-infected mice were monitored for 45 days (C), and differences between the immunized groups and the infection control were compared at 38 dpi (D). Data are presented as mean±SD. **P<0.01, ***P<0.001 and ****P<0.0001.
Noticeable differences in body weight were also observed. Unimmunized mice underwent gradual weight loss from 20 dpi onward, while immunized mice maintained a normal-range body weight until 41 dpi (Fig. 4C), when a rapid decline in body weight was observed, particularly in AMA1-immunized mice. At 38 dpi, none of the mice in either of the 2 immunization groups experienced substantial weight loss (Fig. 4D).
Discussion
Malaria is an endemic tropical disease that affects a large portion of the world’s population. Although the Food and Drug Administrations of several African nations have approved the use of RTS,S/AS01 and R21/Matix-M vaccines, vaccine efficacy is low. Here, we compared the efficacy of VLP vaccines expressing P. berghei AMA1 or MIC antigens in mice following P. berghei challenge infection. We found that VLPs expressing the MIC antigen conferred better protection than VLPs expressing the AMA1 antigen, indicating their potential as a vaccine candidate.
Both antigens used in this study are factors involved in host cell invasion [23]. Specifically, AMA1 is an invasion ligand secreted by the microneme organelle [24]. Several mechanisms of AMA1 antibody-mediated suppression of parasitic growth have been suggested, including epitope blocking to prevent interaction with other invasion ligands and impairing AMA1 secondary processing, among others [25]. Given that VLP immunization induced a substantial increase in P. berghei AMA1-specific antibodies, we anticipated that this would contribute to preventing parasitic invasion of RBCs. Although reductions in parasitemia were observed to some extent, complete inhibition of P. berghei infiltration into RBCs was not achieved. The underlying cause of this observation requires further elucidation, but it is thought to be an immune evasion mechanism unique to P. berghei. In support of this notion, baculovirus-based vaccine immunization in mice induced antibodies that only conferred protection against P. yoelii but not against P. berghei lethal challenge infection [26]. This immune evasion could also account for the failure of vaccine-induced antibodies against P. berghei MIC to completely inhibit parasite invasion. Although speculative, the reason why MIC VLPs elicited better protection than AMA1 VLPs may be partially due to the intrinsic nature of the microneme as an organelle.
Many findings reported in this study are consistent with our previous findings. Immunization of mice with recombinant vaccinia virus (rVV) expressing P. berghei AMA1 or MIC conferred protection, and MIC generally conferred better protection than AMA1 [27]. Similar to MIC VLPs, the rVV-MICs ensured significant induction of blood CD4+ T cells and enhanced the frequency of ILN GC B cells. rVV-MIC immunization also conferred a longer survival time compared with rVV-AMA1 immunization, despite the 2 groups having similar parasitemia burdens. Consistent with this finding, in the present study, mice immunized with MIC VLPs underwent substantially less body weight loss after challenge infection. Furthermore, compared with the AMA1 VLP group, the parasite burden at 45 dpi was markedly reduced in mice receiving MIC VLPs. On the basis of these consistent results, it is highly probable that the MIC antigen is more immunogenic than the AMA1 antigen, but the underlying cause of this phenomenon remains unknown. A factor that may have contributed to this difference in immunogenicity is the IFN-γ level. In studies involving the rodent malaria parasite P. chabaudi, loss of protection was associated with diminished levels of splenic IFN-γ, and this cytokine is necessary for optimal immune cell priming [28,29]. Because mice immunized with MIC VLPs exhibited greater levels of IFN-γ than those immunized with AMA1-VLP, it can be argued that the MIC antigen was more effective at immune cell priming than the AMA1 antigen, which could have contributed to the discrepancies in body weight loss and parasitemia.
Regardless of their pro- or anti-inflammatory nature, cytokines are double-edged swords: they can either be protective or exacerbate disease progression. This is especially true for major inflammatory cytokines, such as IFN-γ and TNF-α, whose function remains controversial. IFN-γ has been reported as essential for parasite clearance [30], whereas another study found that IFN-γ and TNF-α were associated with cerebral malaria development following P. berghei infection in mice [31]. Interesting findings have been reported for IL-10, but its role and involvement in severe malaria are still under debate. For example, IL-10 was reported to reduce parasite sequestration and prevent experimental cerebral malaria development [31,32], whereas other studies documented the association of IL-10 with severe malaria and parasitic growth promotion [33–35]. Furthermore, cytokine profiles from hospitalized patients with malaria revealed that individuals with high levels of IL-10 were prone to developing nosocomial infections [36].
In this study, although vaccine-induced changes in IFN-γ levels were negligible, they contributed to suppressing TNF-α production. Furthermore, IL-10 levels in VLP-immunized mice were lower than the levels in naïve+challenge controls, with significantly less parasite burden. Because remarkably increased levels of TNF-α, IFN-γ, and IL-10 in response to P. berghei infection in Kunming mice have been reported [37], enhanced production of these 3 cytokines was expected. Given these findings, it is plausible that the VLP vaccines conferred protection by preventing the buildup of cytokines to levels that could detrimentally affect the host. Although unrelated to vaccines, cytokine profiles from coinfection studies involving P. berghei also reported similar findings. Coinfecting mice with Babesia microti with P. berghei resulted in markedly reduced IFN-γ and TNF-α levels compared with P. berghei infection alone, and survival was also significantly increased in the coinfection group [38]. Similarly, administering Bifidobacterium longum prior to P. berghei infection significantly diminished IFN-γ and TNF-α production, leading to a significant reduction in parasitemia [39].
In conclusion, we demonstrated that MIC VLPs were effective and conferred adequate protection against P. berghei challenge infection in BALB/c mice. Compared with mice receiving the AMA1 VLPs, the MIC VLPs were more effective, as evidenced by lower levels of parasitemia and body weight loss. Although further optimization is necessary, the MIC VLP presented in this study could be a promising malaria vaccine candidate.
Supplementary Information
Acknowledgment
This study was financially supported by the Core Research Institute (CRI) Program, the Basic Science Research Program through the National Research Foundation of Korea (NRF), Ministry of Education (NRF2018R1A6A1A03025124).
Notes
Conceptualization: Quan FS
Formal analysis: Kim MJ, Chu KB, Quan FS
Investigation: Kim MJ, Chu KB, Yoon KW, Kang HJ, Lee DH, Quan FS
Project administration: Quan FS
Resources: Moon EK, Quan FS
Supervision: Quan FS
Writing – original draft: Kim MJ, Chu KB, Quan FS
Writing – review & editing: Chu KB, Moon EK, Quan FS
The authors declare no conflict of interest related to this study.