Mucosal immunity against parasitic gastrointestinal nematodes
Article information
Abstract
The last two decades witnessed significant advances in the efforts of immunoparasitologists to elucidate the nature and role of the host mucosal defence mechanisms against intestinal nematode parasites. Aided by recent advances in basic immunology and biotechnology with the concomitant development of well defined laboratory models of infection, immunoparasitologists have more precisely analyzed and defined the different immune effector mechanisms during the infection; resulting in great improvement in our current knowledge and understanding of protective immunity against gastrointestinal (GI) nematode parasites. Much of this current understanding comes from experimental studies in laboratory rodents, which have been used as models of livestock and human GI nematode infections. These rodent studies, which have concentrated on Heligmosomoides polygyrus, Nippostrongylus brasiliensis, Strongyloides ratti/S. venezuelensis, Trichinella spiralis and Trichuris muris infections in mice and rats, have helped in defining the types of T cell responses that regulate effector mechanisms and the effector mechanisms responsible for worm expulsion. In addition, these studies bear indications that traditionally accepted mechanisms of resistance such as eosinophilia and IgE responses may not play as important roles in protection as were previously conceived. In this review, we shall, from these rodent studies, attempt an overview of the mucosal and other effector responses against intestinal nematode parasites beginning with the indices of immune protection as a model of the protective immune responses that may occur in animals and man.
INTRODUCTION
Gastrointestinal (GI) nematode parasites are important causes of disease in humans and domestic animals. In humans, species belonging to the genera Ascaris, Ancylostoma, Necator, Strongyloides and Trichuris, all of which are known as soil-transmitted parasites, infect approximately one billion people worldwide and are believed to cause one million deaths annually, with prevalence in some endemic areas in developing countries approaching 100% especially in children (Kightlinger et al., 1995; Finkelman et al., 1997). Since infections tend to be chronic, it is particularly damaging to severely infected children, causing anaemia, growth retardation, impaired cognitive function and lowered educational achievement (Cooper and Bundy, 1988; Nokes et al., 1992; Guyatt, 2000). In addition, infections also cause impaired physical fitness, reduced productivity and low earning potential in adults as a result of high morbidity due to iron-deficiency anaemia and wasting (Guyatt, 2000) (Fig. 1). Parasitic gastroenteritis (PGE), caused by species of the genera Bunostomum, Cooperia, Haemonchus, Nematodirus, Oesophagostomum, Ostertagia, Teladorsagia and Trichostrongylus, is a major problem in farm animals worldwide where it can cause significant production losses and, in heavy infections, death of the host (Balic et al., 2000; Claerebout and Vercruysse, 2000). Control measures are expensive. For instance, based on 1994 sales figures in Britain alone, it was shown that control of PGE costs the livestock industry about one thousand million pound sterling (£1000 million) annually (Newton and Munn, 1999). At present, the control of PGE in animals relies heavily on repeated use of anthelmintic drugs to cure existing infections, but increasing consumer concerns regarding drug residues in animal products and the environment and, the emerging development of resistance by the parasites against anthelmintic drugs in livestock, including the latest family of ivermectin drugs, all highlight the imperative need to seek and adopt more natural and alternative control strategies for the future (Coles, 1998; Newton and Munn, 1999; Vercruysse and Dorny, 1999; Balic et al., 2000). Similarly, control of GI nematode parasitism in humans relies on anthelmintic chemotherapy and, coupled with efficient primary health care and effective public sanitation, human GI nematode parasitism can be effectively eliminated (Finkelman et al., 1997). However, these measures are also expensive and difficult to achieve and sustain in most under-developed and developing countries where GI nematode parasitism remains endemic and intractable. In both cases, immunological intervention through vaccination has been viewed as a feasible control alternative (Emery et al., 1993). In fact, throughout much of the just ended century, researchers intensively studied systemic and mucosal host immune responses to the infections as well as various parasite antigens with the teleologic aim being the development of an effective vaccine as a more cost effective, practical and innovative therapeutic intervention. Accordingly, a great deal of information has been generated on various aspects of immune responses against GI nematode parasites. It is clear, mostly from rodent model studies, that significant protective immunity can be generated following natural and experimental infections. But this not withstanding effective vaccines are yet to be developed for the major GI nematode species. This can be attributed to the earlier difficulties in precise definition and understanding of the various underlying immune mechanisms involved in parasite rejection. Based on the rodent model studies the situation is different now and the next decade aught to see an accelerated advance towards vaccine development. However, although some similarities occur between effector responses in rodent models and some studies in livestock, care must be taken in extrapolating from one host-parasite system to another as differences between host species, nematode species and, in the localization of particular parasites within the host gut mucosa, have important consequences for the interaction between the parasite and the host's gut immune systems and on the type of effector mechanism that is expressed (Nawa et al., 1994; Claerebout and Vercruysse, 2000; Balic et al., 2000).
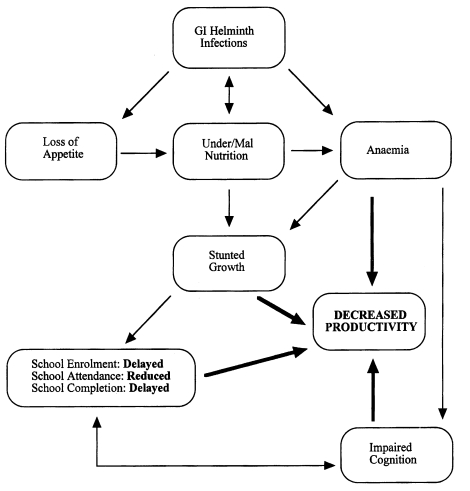
A schematic representation of the interaction between GI nematode infections and under/malnutrition in the genesis of childhood anaemia and the effects on growth, cognitive ability and the ultimate decreased productivity in adulthood (Adapted and modified with permission from Guyatt, 2000).
INDICES OF MUCOSAL IMMUNITY AGAINST GI NEMATODE PARASITES
Immunity against gastrointestinal nematodes can be manifested as expulsion of adult parasites and reduction in worm length, decrease in female worm fecundity, failure of infective larvae to establish and arrested development of larvae.
Expulsion of adult worms
Rapid expulsion of adult worms following primary infections occurs in most rodent nematodes but the phenomenon is uncommon in large animals and man (Behnke et al., 1992). The only exception is Nematodirus species in which adult worms from primary infections of sheep with N. battus were expelled in periods ranging from 18-21, 24-28, upto 72 days post infection (p.i.) depending on the dose of infective larvae given (Balic et al., 2000). Most of the work on rapid expulsion of adult nematodes following primary infections have been in rodents using T. spiralis, N. brasiliensis, Strongyloides species and T. muris. Each of these parasites occupies a different niche in the gut and, as will be reviewed subsequently, evokes slightly different effector mechanisms; thus important differences exist in the ability of the rodent hosts to expel a primary infection with each of them (Else and Finkelman, 1998). T. spiralis, N. brasiliensis, S. ratti and S. venezuelensis all have tissue migratory larval stages and their adults live in the small intestinal lumen from where they are expelled within 2-3 weeks after primary infection (Ogilvie and Hockley, 1968; Miller, 1984; Wakelin and Lloyd, 1976; Nawa et al., 1985; Sato and Toma, 1990a,b). T. muris on the otherhand occupies a niche in the large intestine where it induces syncitium formation and lives partially or completely within the intestinal epithelium and the ability to expel the adult in a primary infection is genetically determined (Wakelin, 1975, Else and Wakelin, 1988). Thus, in some strains the worms are eliminated before they reach sexual maturity and produce eggs while in others a proportion fails to do so and allows the parasites to mature and establish a chronic infection (Wakelin, 1987).
Reduction in worm length
Observations on the changes in morphology of GI nematodes as an index of protective immunity have largely described reduced size (stunting) of adult nematodes although the loss of vulval flap in some adult female worms have been documented (Balic et al., 2000). Most of the studies of immune stunting of adult nematodes in rodent models have been done with T. spiralis although the evidence of stunting exists for H. polygyrus and S. ratti. Mice immunized by primary T. spiralis infection followed by treatment prior to the production of newborn larvae and then challenged with infective larvae showed stunting of the adults as well as earlier expulsion with 95% reduction in the number of muscle larvae which encysted following the challenge (James and Denham, 1975). A similar observation was made by DeVos et al. (1992) in challenge T. spiralis infection in mice. Although they recorded a dose-dependent response, with 28% reduction in the size of adult worms recovered from mice primed and challenged with 10 larvae each and 35% reduction in those from mice primed and challenged with 150 larvae each. In addition, vaccination of mice with various T. spiralis antigen preparations results in protective immunity that also induces stunting of adult worms following challenge (Grencis et al., 1986; Goyal and Wakelin, 1993; Boulos et al., 1993). Adult worms arising from challenge infections of mice that had previously experienced one or more infections of H. polygyrus or from naive mice that had received immune serum prior to challenge were stunted and anaemic with female worms being more severely affected than males (Ey, 1988). Similarly, adoptive transfer of immune mesenteric lymph node cells (MLNC) induced reduction in adult worm size in recepient rats challenged with S. ratti (Moqbel and Wakelin, 1981) and Uchikawa et al. (1989) showed a dose dependent reduction in worm length following single and repeated inoculations of rats with S. ratti. Stunting of adult worms as a result of acquired immunity has also been reported for the livestock parasites Cooperia spp., H. contortus, O. ostertagi, and Trichostrongylus spp. (Balic et al., 2000; Claerebout and Vercruysse, 2000).
Reduction in female worm fecundity
Immune-mediated reduction in female worm fecundity is a very important epidemiological factor and in sheep has been implicated as a major regulatory force for GI nematode populations (Stear et al., 1997). It has been suggested that reduced female worm fecundity can also be as a result of density-dependent intraspecific parasite competition although data on the role of density dependence are conflicting (Balic et al., 2000). Depending on the species of parasite and animal model, reduction in female worm fecundity as a result of developing or acquired immunity can be measured either by reduced faecal egg output, number of eggs in-utero or number of newborn larvae/female worm. In rodent models and ruminants, the first evidence of developing protective immunity to a primary infection and acquired protective immunity following challenge infection is usually a decreasing and no egg output in faeces respectively. Although faecal egg count is the only parasitological parameter of immunity that can be obtained sequentially and regularly in the same animal in the course of an infection, it does not strictly reflect the fecundity of the female worm population (Claerebout and Vercruysse, 2000) as a lot of other factors may affect the faecal egg count. However, a very good correlation between faecal egg counts and the number of eggs in utero was found in C. oncophora (Claerebout and Vercruysse, 2000) and O. circumcincta (Stear et al., 1995) infections in calves and sheep respectively. In rodents infected with Strongyloides or N. brasiliensis faecal egg count as an index of immunity is feasible only in a primary infection as the immunity that develops is very strong and ablates any challenge infection prior to the enteric stage or before maturation and egg production if any larva reaches the gut. Several studies in our laboratory have shown that in such primary infections, development of immune-mediated effect on the female worms is usually manifested as decreasing number of eggs in faeces followed by adult worm expulsion. However, because the decrease may also be related to a gradual decrease in the number of female worms due to expulsion, determining the number of eggs in utero is a better index of decreased fecundity, as shown in S. ratti infection in rats immunized either by adoptive transfer of MLNC (Moqbel and Wakelin, 1981) or by repeated infections (Uchikawa et al., 1989). Similarly, the number of in utero eggs/female was significantly less in euthymic than hypothymic (nude) mice on day 9 after infection with S. venezuelensis (Sato and Toma, 1990a). In BALB/c mice infected with either S. ratti or S. venezuelensis, the fecundity of both species was significantly decreased respectively from 6 and 5 in utero eggs/female worm on day 6 p.i. to 0.8 and 1 in utero eggs/female worm on day 10 p.i. (Sato and Toma, 1990b). Conversely, female worms are significantly more fecund in IL-5 knockout than IL-5 sufficient mice infected with S. ratti (Ovington et al., 1998), although this result was based on dividing the total eggs+larvae by the total number of adult worms. Several workers have demonstrated reduced fecundity of female worms in T. spiralis following infection- and/or vaccine antigen-induced immunity as measured by decrease in the number of newborn larvae/female worm (Wakelin and Wilson, 1977; Kennedy and Bruce, 1981; Grencis et al., 1986; DeVos et al., 1992; Goyal and Wakelin, 1993). On the otherhand, in contrast to decreased fecundity in vaccinated mice, suppression of either vaccine- or infection-induced immunity by super infection with Trypanosoma brucei either at the time of primary T. spiralis infection or at the time of primary vaccination with muscle larva antigen followed by T. spiralis challenge resulted in significantly increased female worm fecundity (Onah and Wakelin, 1999, 2000). Reduction in female worm fecundity has also been described for H. polygyrus (Urban et al., 1991b).
Failure of infective larvae to establish and arrested development of larvae
A major manifestation of acquired immunity to GI nematodes is failure of infective larvae to establish and mature to adults in the gut. This phenomenon has been described both in rodents and ruminants. In rodents, challenge infections with Strongyloides or N. brasiliensis are usually sterile with no egg output in the faeces nor recovery of adult worms. Studies in our laboratory as well as those of other workers have shown that in immune animals most infective larvae following challenge are destroyed at the tissue migratory stage usually with few larvae surviving the transition from the subcutaneous infection site to the lungs. Even fewer or none at all may migrate from the lungs to the intestine where they fail to thrive and are expelled prior to maturity and egg production (Sato and Toma, 1990b; Korenaga et al., 1991; Dent et al., 1997b; Onah DN, Uchiyama F, Ishiwata K and Nawa Y, unpublished observation). Although resistance to larval establishment is most strongly expressed in secondary and subsequent larval challenges, the fact that in most experimental studies 40% or less of the infecting larval dose in a primary infection finally establishes as adults, indicates that resistance to larval establishment is not restricted to challenge infections. Resistance to larval establishment in primary Strongyloides infections has been attributed to complement (Brigandi et al., 1996), IL-5/eosinophils (Ovington et al., 1998) and granulocytes (Watanabe et al., 2000). Failure of larval establishment has also been demonstrated in many ruminant parasites where the resistance is generally stage-specific, requires homologous challenge for expression but can act against heterologous species present in the same tissue and is usually expressed as rapid expulsion of infective larvae from previously primed hosts (Balic et al., 2000).
Arrested larval development at the L4 stage (hypobiosis of larvae) of GI nematodes in the host mucosa is a common phenomenon in ruminant hosts and is associated with increased resistance of the hosts to the parasites. However, it is also associated with a number of other factors such as seasonal changes, population density of the nematode in the host and strain of the nematode (Balic et al., 2000). Its role is epidemiological as it provides the replacements for expelled or aged adult worm populations and its wide spread occurrence in ruminants suggests that it is a successful survival strategy for the parasites. The phenomenon has not been reported in rodent models.
ANTIGEN PROCESSING AND PRESENTATION AT THE GI MUCOSAL SURFACE
Before reviewing the various protective mucosal immune responses against parasitic GI nematodes, it may be helpful to briefly outline how immune responses to orally acquired antigens may occur, although whether they may also apply to nematodes is not clear. Despite significant advances in the understanding of antigen uptake, processing and presentation in general immune system, our knowledge of these events in the GI tract or other mucosal surfaces is limited (Mayer, 1990). Moreover, the GI epithelium and its mucus are thought to constitute a prominent barrier to antigen uptake in the gut (Russell and Walker, 1990), and unless disrupted, antigen uptake may be minimal (Befus, 1995). However, while antigen uptake in the GI tract is associated with specialized microfold (M) cells (Fig. 2) in the follicle-associated epithelium overlying the Peyer's patches (PP) and other gut associated lymphoid tissues (GALT)(Mayer, 1990; Kelsall and Strober, 1999), antigen presenting cells (APC) such as B cells, dendritic cells, epithelial cells, fibroblasts and macrophages are involved in antigen processing and presentation (Mayer, 1990). Following ingestion, soluble antigens and microorganisms are rapidly taken up and transported by M cells from the intestinal lumen to APC in the subepithelial dome (SED) of the PP where initial cognate interactions between APC and T cells, or T cells and B cells occur (Owen and Jones, 1974; Kelsall and Strober, 1999) (Fig. 2). Detailed review of the sequence of these events can be found in many excellent reviews on the subject (Mayer, 1990; Owen, 1994; Befus, 1995; Kelsall and Strober, 1999; Claerebout and Vercruysse, 2000).
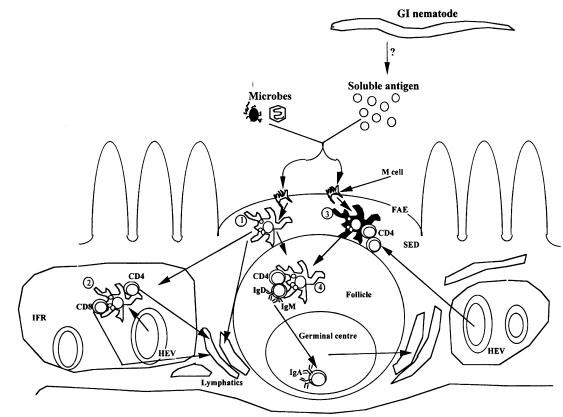
Antigen processing and presentation in the gut mucosa. Possible roles of Peyer's patch (PP) dendritic cells (DC) in the processing of luminal antigens which gain access to the PP across M cells located in the follicle-associated epithelium (FAE). Immature DC in the subepithelial dome, SED (1) acquire antigens, such as microbes via phagocytosis, and soluble antigens via pinocytosis. As these DC differentiate during movement to the interfollicular region (IFR), acquired antigens are processed and peptides are expressed in association with MHC class I and II antigens. In addition, adhesion molecules, such as intercellular adhesion molecule-1 (ICAM-1) and costimulatory molecules, such as B7-1 (CD80), B7-2 (CD86), and CD40, are upregulated, and the differentiation antigens M342 and NLDC-145 are expressed at high levels. In the IFR (2) they stimulate resident CD4+ and CD8+ T cells that have gained entry into the PP across high endothelial venules (HEV) located in the IFR, or these DC move into draining lymphatics, where they traffic to the mesenteric lymph nodes (MLN). A second possibility is that less differentiated DC in the SED process and present antigens to CD4+ T cells at this site (3) or after migration into the follicle (4), resulting in the induction of T cells with a phenotype that is unique to the PP, such as one producing transforming growth factor-β (TGF-β) and/or IL-10. In the follicule, such T cells would be ideally positioned to provide help for switching to IgA, a process that is then completed in the germinal centre. Following IgA switch and affinity maturation, B cells rapidly migrate from the PP to the MLN via efferent lymphatics, and finally to the lamina propria where they undergo terminal differentiation into plasma cells. It is however, not clear whether these possibilities are also applicable to the processing and presentation of nematode antigens in the gut mucosa (Adapted with permission from Kelsall and Strober, 1999).
There is a dearth of studies on the uptake, processing and presentation of helminth antigens in the GI tract or elsewhere, thus there is currently no solid in vivo proof of the capacity of any of the above cells to be involved in nematode antigen presentation in the gut (Befus, 1995, Miller, 1996a). No relationship between M cells and any intestinal helminth has been noted to date but it is thought that perhaps, they may provide some physical or chemical signals which helminths utilize either for site selection or as other microenvironmental clue for survival (Befus, 1995). However, although there is limited information on the nature of the antigens of the abomasal nematode parasite of ruminants Ostertagia (Claerebout and Vercruysse, 2000) and it is not known which of its antigens elicit protective immune responses (Hilderson et al., 1995), there is an evidence that soon after the infection of calves with O. ostertagi, worm antigens were presented to the host cells in the abomasal lymph nodes (Gasbarre, 1997). But in general, it remains to be determined which nematode antigens are relevant in the antigen handling mechanisms, the pathway(s) of their uptake, types of APC utilized and the nature of processing and presentation. In fact, whether or not the remarkable predominance of IgE, mast cell and eosinophil responses in GI nematode infections can be directly attributed to the pathways of uptake, processing and presentation of worm antigens, or to other characteristics of the host-parasite relationship remains to be established (Befus, 1995).
EFFECTOR MECHANISMS IN THE GI MUCOSA AGAINST PARASITIC NEMATODES
There is diverse information concerning the generation of immune and inflammatory responses in the gut mucosa (Lin and Befus, 1999; Kelsall and Strober, 1999). However, investigations of effector mechanisms in intestinal nematode infections have focused on more limited areas such as the types of antigen-specific cells which can transfer immunity, mast and goblet cell responses and the role of selected chemical mediators, including mucus. In general, effector mechanisms against GI nematodes appear to involve antigen-specific T cell responses which induce antibody response and inflammatory changes, with the release of a plethora of chemical mediators ultimately leading to the expulsion of the worms (Befus, 1995). This section will review some of the information available in these areas.
T cells and their role in protection
Several studies with different nematode species have demonstrated that protective immunity against GI nematodes is T cell-dependent. The foundation for this in immunoparasitology was laid by the works of DiNetta et al. (1972) and Larsh et al. (1972) using T. spiralis infections in mice. Independently these authors reported that treatment of mice with anti-thymocyte serum resulted in delayed expulsion of adult T. spiralis worms. These were to be confirmed and extended by the use of congenitally athymic 'nude' mice and rats infected with T. spiralis (Walls et al., 1973; Ruitenberg and Elgersma, 1976; Ruitenberg et al., 1977; Vos et al., 1983). These studies in athymic animals have been corroborated by work with other nematode species such as N. brasiliensis (Mitchell, 1978; Mckay et al., 1995), Nematospiroides dubius (Heligmosomoides polygyrus) (Prowse et al., 1978), S. ratti (Abe and Nawa, 1988) and S. venezuelensis (Sato and Toma, 1990a).
Adoptive transfer experiments and/or in vivo depletion of CD4+ T cells by cytotoxic anti-CD4 monoclonal antibody (mAb) treatments have demonstrated that CD4+ helper T cells play a central role in the T cell-dependent protective immunity against GI nematode parasites (Finkelman et al., 1997; Else and Finkelman, 1998). Using accurate cell sorting and adoptive transfer of immune cells it was demonstrated that CD4+ T cells were the T cell subset involved in the conferement of immunity against challenge infection with T. spiralis in mice (Grencis et al., 1985). Also, thoracic duct CD8-OX22- (presumably CD4+) lymphocytes collected from rats 3 days after infection with T. spiralis conferred immunity against the adult worms in naive rats (Wang et al., 1990). Depletion of CD4+ but not CD8+ cells by mAb treatment at the time of N. brasiliensis infection in mice blocked spontaneous elimination of adult worms as well as polyclonal IgE responses (Katona et al., 1988; Abe et al., 1994) and, was shown to be effective only against the intestinal phase of the infection (Khan et al., 1995). Primary H. polygyrus infection in mice results in chronic infection characterized by faecal egg production for several months. Evidence that protective immunity against H. polygyrus in mice is CD4+ T cell-dependent came from the fact that during a primary infection, mice treated with rat anti-mouse CD4+ mAb had increased faecal egg production which was primarily due to an increase in female worm fecundity (Urban et al., 1991a). It was further shown that if a primary infection was cleared by anthelmintic drug treatment a protective response against challenge developed which reduced adult worm establishment following challenge by up to 80% and their fecundity by more than 90%. This secondary protective response was completely abrogated by anti-CD4 but not anti-CD8 mAb treatment (Urban et al., 1991a). Also following mAb treatment, the expulsion of T. muris was inhibited in anti-CD4 treated mice but no evidence of suppressed immunity was observed in the anti-CD8 treated mice (Koyama et al., 1995).
Cytokine responses
The discovery in mice that CD4+ T cells can be segregated into two distinct T helper subsets, Th1 and Th2, based on their cytokine secretion profiles (Mosmann et al., 1986) provided the basis for subsequent understanding of the underlying T cell regulatory mechanisms controlling resistance to GI nematode infections. Th1 cells produce gamma-interferon (IFN-γ), interleukin 2 (IL-2) and alpha-lymphotoxin (LT-α), whereas Th2 cells secrete IL-4, IL-5, IL-6, IL-9, IL-10 and IL-13, among others. Products of Th1 cells negatively regulate the Th2 cells and vice versa (Mosmann and Coffman, 1989). Which T cell subset gains predominance over the other in an immune response following parasite infection depends on factors such as the type of APC, co-stimulatory molecules, the nature and dose of parasite antigen and, perhaps more importantly, the immediate cytokine environment the T cell experiences at the time of antigen presentation (Grencis, 1996; Constant and Bottomly, 1997). The predominant immune responses that are typical of nematode infections are all controlled by Th2 cytokines (Finkelman et al., 1991), but of all the Th2 cytokines those that have been associated with resistance to various nematode parasites include IL-4, IL-5, IL-9, and IL-13.
The most compelling evidence for the importance of IL-4 in resistance to GI nematode infections came from studies with H. polygyrus and T. muris infections in mice (reviewed by Finkelman et al., 1997; Else and Finkelman, 1998). Anti-IL-4 or anti-IL-4 receptor (IL-4R) mAb treatment was shown to block the immune-mediated expulsion of H. polygyrus challenge infection in mice (Urban et al., 1991b). Similarly, BALB/k mice are normally resistant to T. muris infection but when treated with anti-IL-4R mAb (which also blocks IL-13 receptor), host protective immunity against primary T. muris infection is abrogated, resulting in the establishment of a chronic infection in which Th1 responses predominate while Th2 responses are down-regulated (Else et al., 1994). Furthermore, treatment of mice with an IL-4 complex (IL-4C), obtained by mixing IL-4 and neutralizing anti-IL-4 mAb, which prolongs the in vivo half life of IL-4 by protecting it from degradation and excretion, was shown to be effective in enhancing worm expulsion and in curing even established chronic infections of H. polygyrus (Urban et al., 1995) and T. muris (Else et al., 1994). Further evidence for the significance of IL-4 in protective immunity against H. polygyrus and T. muris was obtained in experiments using IL-4 gene knockout (KO) mice (Finkelman et al., 1997; Bancroft et al., 1998; Artis et al., 1999). The role of IL-4 in protective immunity has also been demonstrated in normal and immune deficient mice infected with N. brasiliensis. IL-4 treatment terminated N. brasiliensis infections in severe combined immunodeficiency (SCID) mice and in anti-CD4 mAb-treated BALB/c mice (Urban et al., 1995). However, both anti-IL-4 mAb-treated mice and mice lacking the IL-4 gene expel N. brasiliensis normally (Madden et al., 1991; Lawrence et al., 1996), suggesting that IL-4 is sufficient but not critical for expulsion of N. brasiliensis.
The role of IL-5 in protective immunity to GI nematode parasites is not very clear, largely because it has been difficult to demonstrate anti-parasite effects of eosinophils in vivo, the production of which during parasitic infections depends on IL-5, although in vitro killing by eosinophils is easily demonstrated (Butterworth et al., 1974; Korenaga and Tada, 1994). Thus, the involvement of IL-5/eosinophilia in resistance to intestinal helminthiasis remains an unresolved controversial issue. Several studies involving IL-5 transgenic mice or mice treated with neutralising mAb against IL-5 prior to infection with various species of helminths indicate that IL-5 may enhance resistance to some but not all parasites and that some parasites may even fair better in IL-5 transgenic animals (reviewed by Onah et al., 2001). For instance, following a primary T. spiralis infection higher numbers of muscle larvae were established in IL-5 transgenic mice than their non-transgenic counterparts whereas in transgenic mice infected with N. brasiliensis, few larvae survived to reach the lung and even fewer finally made it to the intestines where they failed to thrive and produce eggs (Dent et al., 1997b).
Although limited, there is an evidence that IL-9 which in humans and mice has been shown to potentiate IL-4-induced Th2-type antibody production (Dugas et al., 1993; Petit-Frere et al., 1993), also plays a role in resistance against GI nematode parasites. First suggestive evidence for the role of IL-9 in protection came from studies with T. muris infections in BALB/k and B10.BR mice which differ in an absolute fashion in their ability to resist the infection. Whereas the former mice strain expels T. muris relatively rapidly, the latter are unable to expel the parasite before the infection reaches patency. When MLNC obtained from T. muris-infected BALB/k and B10.BR mice were stimulated in vitro with Con A and analyzed for Th1 and Th2 cytokine production, MLNC from BALB/k mice were shown to produce elevated levels of IL-9 with little amount of IFN-γ whilst MLNC from B10.BR mice produced large amounts of IFN-γ in the relative absence of IL-9 (Else et al., 1992a). When BALB/k mice were rendered susceptible to T. muris by hydrocortisone treatment their MLNC produced significantly higher amounts of IFN-γ and low levels of IL-9 than MLNC from untreated infected mice whereas, in B10.BR mice immunized with T. muris E/S antigen there was accelerated expulsion of T. muris with elevated production of IL-9 and no IFN-γ by MLNC (Else et al., 1992b). Studies with mice in which IL-9 was elevated in vivo prior to T. muris infection and with IL-9 transgenic mice which constitutively overexpress IL-9 showed that the animals displayed an extremely rapid, but immune-mediated, expulsion of the parasite accompanied by pronounced intestinal mastocytosis and enhanced production of IgG1 and IgE while the neutralization of IL-9 by treating normally resistant C57BL/6 mice with neutralizing anti-IL-9 coupled to ovalbumin rendered them susceptible (Faulkner et al., 1998; Richard et al., 2000). The importance of IL-9 in immune-mediated protection against H. polygyrus (Behnke et al., 1993, Wahid et al., 1994) and T. spiralis (Behnke et al., 1993; Faulkner et al., 1997; Else and Finkelman; 1998) has also been demonstrated.
As mentioned above, although IL-4 plays a central role in Th2-dependent protective immunity against GI nematodes, a couple of studies showed that both IL-4 KO mice and mice treated with anti-IL-4 mAb expel N. brasiliensis normally (Madden et al., 1991; Lawrence et al., 1996), suggesting an independence of the process on IL-4 and that another Th2 mediator may be required for worm expulsion. However, a number of recent studies reveal that both IL-4, and a related cytokine IL-13, play protective roles in N. brasiliensis, T. muris and T. spiralis infections in mice, but show parasite-specific differences in their relative importance or redundancy (Finkelman et al., 1999) (Table 1). Studies that compared the kinetics of expulsion of N. brasiliensis in wild type, IL-4 KO and IL-13 KO mice revealed a distinct and critical role for IL-13 in worm expulsion since only IL-13 KO mice failed to clear the infection (McKenzie et al., 1998). IL-13 shares some, but not all, biological activities with IL-4 (Finkelman et al., 1999), and as mentioned earlier, anti-IL4R mAb treatment blocks both IL-4 and IL-13 which would suggest that the IL-4 independent worm expulsion is not mediated by IL-13. It is therefore, rather paradoxical that whilst infected mice injected with recombinant IL-4 or IL-13 can expel the worm, as does IL-4 KO mice, IL-13 KO mice cannot. This apparent paradox is now explained by the observation that IL-4 receptor alpha chain-deficient (IL-4Rα KO) and signal transducer and activator of transcription molecule 6-deficient (STAT6 KO) mice, as well as mice treated with anti-IL-4R mAb all fail to expel a primary N. brasiliensis infection (Urban et al., 1998). Unlike IL-4, IL-4Rα chain is required for N. brasiliensis expulsion; furthermore, Th2 development during the infection (as indexed by cytokine production, expression of the transcription factor GATA-3 and the cell surface receptor CD30) was more severely impaired in IL-4Rα KO than in IL-4 KO mice (Barner et al., 1998). These authors also demonstrated that the injection of recombinant IL-13 induced worm expulsion in otherwise incompetent, B and T lymphocyte deficient RAG2 KO mice which suggests that IL-13 regulation of Th2 responses to nematode infection requires IL-4Rα. There is an evidence that IL-4 and IL-13 share receptor components (Fig. 3) and that IL-13 binds to a receptor which contains the IL-4Rα chain (Zurawski et al., 1993,1995; Obiri et al., 1995; Smerz-Bertling et al., 1995). In addition, only IL-4 and IL-13 are currently known to induce STAT6 activation via the IL-4Rα chain, so taken together the above studies show that IL-13 mediates resistance to N. brasiliensis in the absence of IL-4 (Else and Finkelman, 1998). Indeed, when immunocompetent mice infected with N. brasiliensis were treated with a soluble IL-13Rα 2-human IgGFc fusion protein (sIL-13Rα 2-Fc), worm expulsion was dramatically inhibited with fecund adults still present on day 16 p.i., and similarly treated IL-4 KO mice harboured even higher numbers of more fecund parasites. These together suggest that both IL-4 and IL-13 contribute to N. brasiliensis expulsion through an IL-4Rα-dependent and STAT6-dependent mechanism with IL-13 being quantitatively the more important cytokine (Else and Finkelman, 1998; Finkelman et al., 1999).

Summary of the outcome of various forms of cytokine manipulation in mice infected with GI nematode parasites
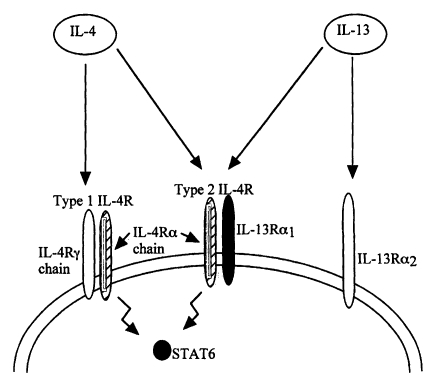
Schematic representation of IL-4 and IL-13 receptors. Anti-IL-4 mAb treatment blocks both IL-4 and IL-13 because both cytokines share (bind to) the type 2 IL-4R and activate STAT6 through this receptor. Thus, in the absence of IL-4, IL-13 is able to mediate worm expulsion via the IL-4Rα chain (Adapted and modified with permission from Finkelman et al., 1999).
Recent studies also revealed a critical role for IL-13 in protection against T. muris infection (Bancroft et al, 1998) and that the process is tumour necrosis factor alpha (TNF-α)-dependent since it is impaired by blocking TNF-α and enhanced by administration of recombinant TNF-α (Artis et al., 1999). In addition, IL-13-mediated T. muris expulsion is independent of B7 costimulation but IFN-γ sensitive. Thus, in mice infected with T. muris, blocking B7 ligand interaction by treatment with murine CTLA4-Ig fusion protein inhibited worm expulsion and enhanced IFN-γ production but did not inhibit IL-13 production (Urban et al., 2000a). When both B7 and IFN-γ were blocked, protective immunity was restored suggesting that the B7-independent protective immune response to T. muris is mediated by IL-13. This was confirmed by subsequent experiments in which additional administration of A25 to further block IL-13 in infected mice in which both B7 and IFN-γ had been blocked resulted in dramatic increase in worm burdens similar to but above those seen in T. muris-infected mice treated with CTLA4-Ig alone (Urban et al., 2000a). Furthermore, these authors demonstrated that IL-4/IFN-γ double knockout mice showed enhanced protection against T. muris infection which was abrogated by IL-13 blockade following A25 administration. While endogenous production of IFN-γ also suppresses T. spiralis expulsion, the production of IL-4 or IL-13 is essential and sufficient to induce spontaneous expulsion of T. spiralis in addition to the expression of IL-4Rα and the associated signaling molecule STAT6, and mastocytosis (Urban et al., 2000b).
Antibody responses
Intestinal nematode infections are typically accompanied by elevated IgE and IgG1 antibody isotypes, which over the years have been favoured as effector molecules in resistance based on the ability to transfer immunity with immune serum. However, passive transfer experiments have provided equivocal results, thus it was observed more than twenty years ago that although immunity can be transferred with immune serum it has proved difficult in an infection to correlate protection with circulating antibody levels (Wakelin, 1978). This statement remains as valid today as it was then. Immunity to adult stage of T. spiralis in mice, as assessed by an acceleration of worm expulsion, was markedly transferred to naive recipient mice but only when the immune serum was transferred along with immune mesenteric lymph node cells taken from the donor mice at day 8 p.i. (Wakelin and Lloyd, 1976). Also in rats, complete destruction of T. spiralis newborn larvae in vivo after passive transfer, as measured by muscle larvae burden, was only evident after exposure to both immune serum and immune cells, and not to either alone (Wang and Bell, 1988). Similarly, rapid expulsion of infective muscle larvae could be transferred to naive adult rats with immune serum collected on day 28 p.i. and thoracic duct lymphocytes (TDL) collected on days 3-5 p.i., but neither serum nor cells could transfer rapid expulsion when given alone, even in large volumes (Ahmad et al., 1990). In the day 28 p.i. serum, the most important Ig isotype was shown to be homocytotropic IgE which when purified could induce rapid expulsion after passive transfer with TDL in as little as 183 µg/rat whereas similarly purified monoclonal IgG of any isotype transferred in amounts of up to 35 mg/rat could not transfer rapid expulsion to rats previously transfused with TDL (Ahmad et al., 1991). In contrast, there is a evidence that immune IgG monoclonal antibodies alone were effective in the transfer of rapid expulsion to neonatal rats (Appleton et al., 1988) and in addition, when primed by prior infection with H. polygyrus instead of immune cell transfer, both IgE and IgG mononclonal antibody isotypes were equally effective in inducing rapid expulsion of T. spiralis in adult rats as well (Bell et al., 1992). Related to this is the fact that high levels of immunity against T. spiralis in mice can be achieved by passive transfer of only immune serum obtained from vaccinated, or from vaccinated and infected mice donors (Robinson et al., 1995; Boulos et al., 1998).
Studies with T. muris also typify the inconsistency in demonstrating an essential role for antibodies in resistance to GI nematodes. Transfer of immune serum on days 0 and 3 p.i. accelerated worm expulsion but delaying serum transfer until days 7 and 8 p.i. failed to enhance expulsion, although immune MLNC accelerated expulsion whether transferred early or late in infection (Wakelin, 1975). Also, the adoptive transfer of highly purified immune CD4+ T cells to SCID mice protected them from primary T. muris infection in the complete absence of an antibody response since they lack functional B or T cell function (Else and Grencis, 1996). Furthermore, whilst immune serum from resistant (B10.BR × B10.G) F1 hybrid mice containing high levels of IgG1 antibody specific for T. muris E/S and other IgG antibodies which recognised two high molecular weight E/S antigens was effective in transferring protection to the non-responsive B10.BR mouse strain (Else et al., 1990), passive transfer of immunity could not be achieved with IgG and IgM mAb although two IgA mAb effectively transferred immunity (Roach et al., 1991). The importance of antibodies in protection against T. muris infection is also questioned by studies with IL-9 transgenic mice which expel T. muris very rapidly in the absence of any detectable parasite-specific IgG antibodies in the serum, though it was reasoned that this may have been because the majority of larvae traversed through the gut so rapidly that insufficient antigen was available for B cell activation and antibody production (Faulkner et al., 1998).
Injection of immune serum alone induced highly specific protective immunity in mice infected with N. dubius (H. polygyrus) (Brindley and Dobson, 1983) but in contrast, considerably greater protection was transferred to recipient mice only when they received both immune serum and immune MLNC (Behnke and Parish, 1981; Williams and Behnke, 1983). A non-essential role for antibody in protective immune responses is also seen in N. brasiliensis infection where mice treated with anti-IgM produce little antibody but retain the ability to expel N. brasiliensis (Jacobson et al., 1977). In the other hand, N. brasiliesnsis-infected STAT6 KO mice generate strong parasite-specific antibody responses yet they are unable to expel the parasites (Urban et al., 1998). Studies of adoptive transfer of immunity in Strongyloides infections also lacked consistency. Resistance to S. ratti infection was transferred with either pooled immune serum or immune MLNC but not with immune spleen cells to mice (Dawkins and Grove, 1981) and with either pooled immune serum or immune serum fraction containing predominantly IgG1 to infected recipient rats (Murrell, 1981). In contrast, serum transfer from immune mice to nude mice failed to confer protection against S. venezuelensis whereas protective immunity was transferred to nude mice that received either normal or immune spleen cells from syngeneic heterozygous thymus-bearing littermates (Sato and Toma, 1990a). In general therefore, there seems to be very little consistent and convincing data to indicate that the antibody represents a principal effector mechanism in resistance to lumen-dwelling gastrointestinal nematodes, although relatively little attention has been paid to local as opposed to systemic antibody production; thus antibodies secreted at gut mucosal surfaces may yet prove to be important in protective immunity against gut dwelling helminths (Else and Finkelman, 1998).
Eosinophils
Significant blood eosinophilia and increase in the number of eosinophils in the GI mucosa (Rothwell, 1989) as well as a marked increase in the levels of IL-5, the Th2 cell cytokine responsible for the generation of eosinophils (Korenaga and Tada, 1994), are prominent features of GI nematode infections. Despite this, the involvement of eosinophils/IL-5 in protective immunity remains controversial as a protective role for them during the infection has not been consistently demonstrated (Table 2). There are evidences that eosinophils kill a variety of parasites in vitro (Butterworth et al., 1975; Kazura and Grove, 1978; Butterworth, 1984; Gransmuller et al., 1987). These studies projected eosinophils as effectors of anti-parasite immunity. However, while the in vitro studies seemed quite convincing, data from in vivo studies have been less clear and convincing. The first suggestive evidence of an eosinophil-mediated protection against a variety of parasites in vivo came from studies of the effects of anti-eosinophil serum administration. Thus, treatment of mice with a monospecific rabbit anti-eosinophil serum (AES) prior to primary infection with the vessle-dwelling helminth Schistosoma mansoni had no effect on the number of schistosomula recovered from the lungs compared to untreated controls (Mahmoud et al., 1975). However, a similar AES treatment prior to challenge abrogated the strong secondary immunity, as seen in untreated immune controls, with highly significant numbers of both schistosomula and adult worms recovered from their lungs and portal system respectively (Mahmoud et al., 1975). In addition, a partial immunity conferred on naive recipient mice by adoptive immune serum transfer was also ablated by the AES treatment (Mahmoud et al., 1975). Similarly, AES treatment in mice infected with T. spiralis had no effect on the spontaneous expulsion of adult worms from the small intestine, but the numbers of larvae in the mucsles were almost doubled by the treatment (Grove et al., 1977). On the otherhand, AES treatment resulted in a striking decrease in the number of intestinal eosinophils and significantly increased the susceptibility of guinea-pigs to both primary and secondary infections with the ruminant parasite T. colubriformis (Gleich et al., 1979).
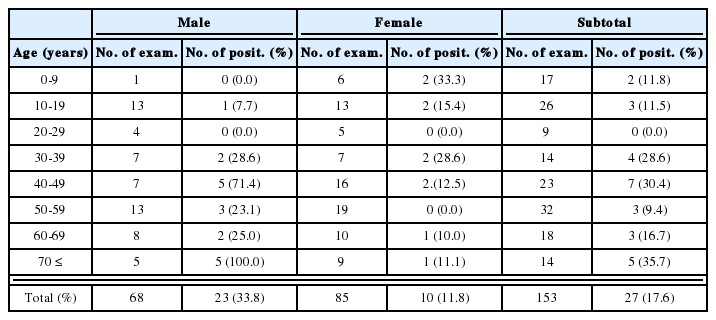
Summary of the protective role of eosinophils in helminth infections as determined by antibody treatment or manipulation of IL-5 and IL-5R
In vivo studies involving the ablation of blood and tissue eosinophlia by anti-IL-5 or anti-IL-5R mAb treatment or by targeted disruption of the IL-5 or IL-5Rα gene (IL-5 or IL-5Rα KO), as well as studies with IL-5 transgenic mice, which constitutively over express IL-5 and over produce blood and tissue eosinophilia, have presented similar conflicting data as above regarding the role of eosinophils as anti-parasite effector cells. Anti-IL-5 or anti-IL-5R mAb treatment resulted in increase in the number of lung larvae recovered from mice following secondary S. venezuelensis infection (Korenaga et al., 1991). Similarly, anti-IL-5 mAb treatment reduced the resistance of immune mice challenged with S. stercoralis (Rotman et al., 1997) and enhanced the survival and numbers of tissue larvae recovered from mice primarily infected with the rat lung worm Angiostrongylus cantonensis (Sasaki et al., 1993) or the filarial parasite Onchocerca lienalis (Folkard et al., 1996). In contrast, following primary or secondary infection, anti-IL-5 mAb treatment had no effect on the number of recovered larvae in mice infected with T. spiralis (Herndon and Kayes, 1992), Toxocara canis (Parsons et al., 1993) and S. stercoralis (Rotman et al., 1996). Similarly, mAb treatment had no effect on the number of adult worm establishment in mice infected with S. venezuelensis (Korenaga et al., 1991), S. mansoni (Sher et al., 1990), S. japonicum (Cheevers et al., 1991), H. polygyrus (Urban et al., 1991b), N. brasiliensis (Coffman et al., 1989; Khan et al., 1995) and T. muris (Betts and Else, 1999). Significantly greater numbers of intracranial larvae were recovered from IL-5Rα KO mice than from their IL-5Rα sufficient controls following primary infection with A. cantonensis (Yoshida et al., 1996; Sugaya et al., 1997) but the number of tissue larvae recovered did not differ between IL-5 KO and their wild type controls following primary T. canis infection (Ovington and Behm, 1997; Takamoto et al., 1997). However, IL-5 KO mice had higher numbers of adult parasites following primary infection with H. polygyrus and S. ratti (Ovington and Behm, 1997) and after primary or secondary T. spiralis infection (Matthaei et al., 1997). Consistent with the mAb treatment and IL-5Rα KO results above, IL-5 transgenic mice are protected against A. cantonensis infection (Yoshida et al., 1996; Sugaya et al., 1997). Similarly, resistance to both the tissue migratory larval and intestinal adult stages of N. brasiliensis is enhanced in IL-5 transgenic mice but in contrast, IL-5 transgenic mice tend to harbour more larval or adult parasites than their non-transgenic littermates following primary or secondary infection with S. mansoni (Freeman et al., 1995; Dent et al., 1997a) and T. spiralis (Hokibara et al., 1995; Dent et al., 1997b) while they fared neither better nor worse than non-transgenic controls following primary or secondary infection with T. canis (Sugane et al., 1996; Dent et al., 1997b). From the foregoing it can be concluded that although eosinophils would appear not to be necessary for immunity to the gut stages of nematodes, they are very effective against the tissue migratory larval stages of at least some but not all helminth parasites (Table 2).
Mast cells
Several studies demonstrated that GI nematode infection is almost invariably accompanied by mucosal mast cell (MMC) hyperplasia (Rothwell, 1989; Miller, 1996b), which may be concomitant with the immune-mediated elimination of the adult parasite following a primary infection (Miller, 1996b). Thus, intestinal mast cells have long been considered as possible effector cells at the mucosal level against GI nematodes (Askenase, 1980; Bienenstock and Befus, 1980; Miller, 1984). However, the period of mastocytosis varies depending on the parasite species and experimental animal host, and does not always coincide with the time of worm expulsion (Lee and Wakelin, 1982; Nawa et al., 1994; Ishiwata et al., 1999). In addition, there are instances of ability to expel worms in the absence of mucosal mastocytosis or, conversely, absence of worm expulsion in the presence of marked MMC hyperplasia (Crowle and Reed, 1981; Nawa et al., 1994; Ishiwata et al., 1999). For instance, mast cell-deficient W/Wv mice, with mutation affecting the tyrosine kinase activity of the stem cell factor (SCF) receptor c-kit, are still able to expel N. brasiliensis (Crowle and Reed, 1981) and, suppression of mucosal mastocytosis by anti-IL-3 and anti-IL-4 mAb treatment during primary N. brasiliensis infection in mice did not prevent worm expulsion (Madden et al., 1991). Similarly, treatment of a resistant strain of mice with anti-IL-3 mAb or with anti-c-kit antibodies did not prevent the expulsion of T. muris (Betts and Else, 1999). On the otherhand, STAT6 KO mice develop a strong intestinal mastocytosis during N. brasiliensis infection but are unable to expel the parasites (Urban et al., 1998), just as primary S. ratti and S. venezuelensis infections persist in Mongolian gerbils and Syrian golden hamsters even when intense mastocytosis was observed (Horii and Nawa, 1992; Horii et al., 1993, Khan et al., 1993a; Shi et al., 1994a, 1995). In addition, faecal egg output in N. brasiliensis-infected Ws/Ws mast cell-deficient rats is reduced when compared with mast cell-sufficient littermates (Arizono et al., 1993) and, suppression of MMC hyperplasia by anti-SCF antibodies treatment in rats infected with N. brasiliensis also significantly reduces faecal egg output by the parasites (Newlands et al., 1995) which together raise the interesting suggestion that mast cells may have some effects that favour the fecundity of N. brasiliensis.
The above not withstanding, the most compelling evidence for the protective role of MMC against GI nematodes comes from studies of the expulsion kinetics of Strongyloides spp. and T. spiralis (Nawa et al., 1994; Miller, 1996b). Several workers had in the past associated MMC with the expulsion of S. ratti (Ishiwata et al., 1999) which was eventually substantiated by studies using mast cell-deficient W/Wv mice as host as well as those that employed repetitive administration of IL-3 to infected animals. When W/Wv mice were infected with either S. ratti (Nawa et al., 1985) or the related parasite S. venezuelensis (Khan et al., 1993b) adult worm expulsion was delayed, but both accelerated worm expulsion and mucosal mastocytosis were completely restored by bone marrow grafting (Nawa et al., 1985). However, when bone marrow-derived cultured mast cells which are phenotypically similar to MMC were adoptively transferred to W/Wv mice, intestinal mastocytosis but not worm expulsion was restored, thus casting a doubt over the role of MMC (Abe and Nawa, 1987a). This doubt was subsequently cleared by the observation that MMC migrated intraepithelially in both S. ratti-infected normal and bone marrow reconstituted W/Wv mice but not in infected W/Wv mice reconstituted with cultured mast cells (Abe and Nawa, 1987b). Subsequently, it was clearly demonstrated that repetitive administration of recombinant IL-3 induced intestinal mastocytosis and enabled hypothymic nude mice to expel primary S. ratti infection (Abe and Nawa, 1988). Moreover, when normal C57BL/6 mice were also treated with IL-3 prior to oral infection with infective larvae of S. ratti recovered from the head of infected rats, worm recovery from the intestine was significantly reduced as early as 6 h after which suggests that MMC are equally effective in preventing worm establishment as in the expulsion of established adults in the intestine (Abe et al., 1993a). In addition, KitW/KitWv, IL-3 KO mice, which are profoundly mast cell deficient, were unable to expel primary S. venezuelensis infection when compared with thier KitW/KitWv, IL-3 sufficient counterparts which expelled all worms by day 18 or 19 after the infection (Lantz et al., 1998). Mastocytosis also represents a major component of the severe inflammatory response provoked in the small intestine of T. spiralis-infected mice and there is an evidence that as in Strongyloides, the expulsion of adult T. spiralis is delayed in W/Wv mice (Alizadeh and Wakelin, 1984). Similarly, antibodies to SCF or c-kit abrogated MMC response and inhibited the expulsion of a primary T. spiralis infection (Grencis et al., 1993; Donaldson et al., 1996). In addition, when IL-9 transgenic mice which exhibit extremely fast expulsion kinetics of T. spiralis are treated with anti-c-kit mAb, worm expulsion is dramatically delayed accompanied by severe depression of intestinal mastocytosis (Faulkner et al., 1997).
In situations where mast cells appear to play an important role in worm expulsion, the precise mechanism of the mediation was less clear. Results of concurrent infection with S. ratti and N. brasiliensis clearly indicated that whereas goblet cells are involved in the expulsion of the latter, but not goblet cells are involved in the expulsion of the former in mice and rats, which suggests that a yet to be defined effector molecule(s) for the expulsion of Strongyloides could be derived from mast cells only (Nawa and Korenaga, 1983; Abe et al., 1992, 1993b). Ironically, the clue to the possible effector molecules in mast cell-mediated adult worm expulsion came from goblet cells in hamsters. Unlike in mice and rats, the expulsion of S. venezuelensis in Syrian golden hamsters is associated with massive goblet cell hyperplasia and production of large quantities of mucin, and not with mastocytosis which although present do not migrate intraepithelially nor does the peak coincide with the time of expulsion (Shi et al., 1994a, 1995). Goblet cell mucins in four different species of hamsters were shown to be highly sulphated but the degree of sulphation determined the rapidity of expulsion. Hamsters that have heavily sulphated goblet cell mucins expelled S. venezuelensis in 2 weeks, whereas those with moderately sulphated mucins expelled in 50 days (Shi et al., 1994b; Ishiwata et al., 1999). These studies suggest that highly sulphated mucins may substitute for mast cell derived effector molecules in the expulsion of Strongyloides in mice and rats. This possibility was strengthened by studies in rats where reserpine-induced sulphated intestinal goblet cell mucin inhibited the establishment of implanted adult S. venezuelensis (Ishikawa et al., 1995). High molecular weight proteoglycans in mast cell granules are highly sulphated and based on the above, it is tempting to propose that sulphated proteoglycans released from mast cell granules upon degranulation may be the effector molecules that prevent the establishment of adult Strongyloides and thus mediate thier expulsion. Our recent studies support this proposal. The deletion of the gene for the γ subunit of Fc receptors results in loss of mast cell functions, including IgE-mediated degranulation and mediator release (Takai et al., 1994). When we infected FcRγ KO and FcRγ sufficient wild type (FcRγ+/+) mice with S. venezuelensis, mastocytosis was similar in both, but whereas FcRγ+/+ controls expelled nearly all the worms by day 13 post infection, the FcRγ KO mice retained the worms for over 25 days (Onah et al., 2000) (Fig. 4). Furthermore, recently it was shown that mast cells contribute to the expulsion of S. venezuelensis from mice by preventing the invasion of the host intestinal mucosa by the adults since treatment with glycosaminoglycans of the type produced by mast cells, such as chondroitin sulphate-A (ChS-A), ChS-E, heparin and dextran sulphate, inhibited in vivo, the binding of S. venezuelensis adhesion molecules to mucosal epithelium and thus the invasion of the gut mucosa by the adults (Maruyama et al., 2000).
Goblet cells
Goblet cell hyperplasia is a feature of many GI nematode infections and the protective function is considered to be mediated through its associated increased mucus production which excludes and traps worms in immune animals (Miller and Nawa, 1979a,b; Lee and Ogilvie, 1980, 1982; Miller et al., 1981) and prevents thier establishment by hindering intimate contact with the gut mucosa (Miller, 1987). Perhaps the strongest evidence of a protective role for goblet cells comes from N. brasiliensis model studies in rats in which there is a chronological association between goblet cell hyperplasia and worm expulsion (Nawa et al., 1994). When rats were concurrently infected with N. brasiliensis and S. ratti, the two parasites were expelled separately; the former in association with goblet cell hyperplasia and the latter with mastocytosis (Nawa and Korenaga, 1983). Furthermore, adoptively transferred immune T cells induced both goblet cell hyperplasia and worm expulsion in rats infected with N. brasiliensis (Miller and Nawa, 1979a), indicating the involvement of T cells in the process. A major break-through in the elucidation of the role of goblet cells in mucosal defence against N. brasiliensis came from simple lectin histochemical staining of jejunal sections which showed changes in the terminal sugars of the mucins produced and secreted by the goblet cells (Koninkx et al., 1988; Ishikawa et al., 1993; 1994; Oinuma et al., 1995). Whereas lectins did not bind to goblet cell mucins in uninfected rats, terminal sugars of goblet cell mucins of N. brasiliensis-infected rats were qualitatively altered and bound strongly to lectins with increase in the number of goblet cells as well as alteration in terminal sugars of goblet cell mucins consistently occurring in association with worm expulsion (Ishikawa et al., 1993; Oinuma et al., 1995). However, it was to emerge that the qualitative changes in goblet cell mucins rather than the quantitative change in goblet cells were more important in the final expulsion of adult N. brasiliensis. When normal (obtained 7 days after primary infection) or immune damaged (obtained 13 days after primary infection) adult N. brasiliensis were implanted intraduodenally into naive or immune euthymic and naive hypothymic (rnu/rnu) rats, normal worms established in euthymic rats before being expelled between days 10-14 in association with goblet cell hyperplasia and alteration in terminal sugars of goblet cell mucins whereas the worms were retained in rnu/rnu rats without significant change in goblet cell responses. In contrast, both strains of rat expelled implanted damaged worms by day 5 but while this occurred in association with goblet cell hyperplasia and alteration in terminal sugars of goblet cell mucins in euthymic rats only the alteration in terminal sugars of goblet cell mucins was observed in rnu/rnu rats (Ishikawa et al., 1993; 1994). Furthermore, dexamethasone treatment completely abolished goblet cell changes in both euthymic and hypothymic rats and suppressed the expulsion of implanted damaged worms. When both strains were primed by implantation of damaged worms to induce goblet cell changes, and challenged 3-5 days later by implantation of normal worms they completely prevented the establishment of the normal worms albeit less effectively in the hypothymic strain (Ishikawa et al., 1994). Apart from N. brasiliensis, goblet cell hyperplasia is also prominent during primary infections of mice with T. spiralis (Garside et al., 1992; Ishikawa et al., 1997) and T. muris (Else and Finkelman, 1998) with a correlation between worm expulsion and peak mucin production observed for the latter parasite.
Gut secretions and motor systems
A substantial number of worms following expulsion from infected hosts are not irreversibly damaged by immune effectors but retain some viability and can reestablish when transferred to a naive host (Kennedy and Bruce, 1981). Therefore, apart from immune responses, parasites may elicit in the hosts some physiological processes which assist in their eviction from the gut prior to thier irreversible damage by gut immune effectors. For instance, increase in water and electrolyte secretions is a common feature and occurs within days or minutes after primary and secondary infections respectively with nematode parasites (Castro et al., 1979; Castro, 1989). This can be interpreted as a host physiological mechanism that assist in parasite eviction (Vallance and Collins, 1998) since secretory diarrhoea, a consequence of chloride (Cl-) secretion, may flush the GI tract of its parasites and/or produce a hostile environment to the parasites (Baird and O'Malley, 1993). Fluid and electrolyte transport is influenced by immunological reactions and, as stated above, is dramatically expressed following challenge. In T. spiralis-sensitized rats, Cl- secretion is mediated via an anti-Trichinella reaction, triggered by IgE and mast cells, which release two preformed Cl- secretagogues (5-hydroxytryptamine [5-HT] and histamine) and leads to local, de novo synthesis of a third, prostaglandin E2 (PGE2) (Castro et al., 1987). The amines work largely through enteric nerves to stimulate Cl- secretion transiently within the first 2 min after antigenic challenge, followed by a second phase of secretion mediated by PGE2 that peaks at about 5 min and wanes over a period of about 20 min (Castro et al., 1987; Castro, 1989). Interestingly, the late but not early phase Cl- secretion could be passively transferred to naive recipient rats with serum containing anti-Trichinella IgE antibodies (Harari et al., 1987, Harari and Castro, 1989) whereas both phases could be passively achieved only when recipient rats were primed by N. brasiliensis infection to induce MMC hyperplasia prior to immune serum transfer (Harari and Castro, 1989).
In addition to the role of fluid and electrolyte secretion, there is a convincing evidence of the involvement of the intestinal motor system in host defence against GI nematode infections. It was demonstrated that increased propulsive forces occurred in extrinsically denervated gut segments of T. spiralis-infected guinea pigs (Alizadeh et al., 1987). Measurements of the correlate of gut motility (i.e. small bowel myoelectric activity) in conscious rats following challenge T. spiralis infection suggest that the in vitro changes in the gut fluid propelling behaviour may also occur in vivo (Palmer and Castro, 1986). The longitudinal muscles of the jejunum of rats infected with T. spiralis developed increased tension by day 6 p.i. (Vermillion and Collins, 1988) as well as hyperplasia and hypertrophy of jejunal smooth muscles (Blennerhassett et al., 1992). The altered muscle contractility was partly attributed to suppression of Na-K ATPase activity in the mucsle (Khan and Collins, 1993). Since it had been shown previously that the alteration in muscle contractility was attenuated in infected rats treated with corticosteroid (Marzio et al., 1990) and in infected athymic rats (Vermillion et al., 1991), it was inferred that the alteration reflected the host's inflammatory response to the infection, rather than a direct effect of the parasite and that the attenuation in athymic rats suggests a T cell dependence and a tangible interface between the immune and motor systems (Vallance and Collins, 1998). The most convincing evidence for the role of the motor system came from works which compared the intestinal mucsle function in a mouse strain (NIH) which expels T. spiralis infection quickly (strong responder) with that in a mouse strain (B10.BR) which expels poorly (poor responder). While intestinal mucsle function prior to infection was similar in both strains, the NIH mice developed significantly greater maximum tension (Vallance et al., 1997) and chronic increase in muscle function (Barbara et al., 1997) than B10.BR mice following T. spiralis infection. As mentioned above, the change in muscle contractility appears to be modulated by T cells, especially CD4+ T cells, since CD4- but not CD8-deficient mice showed reduced muscle contractility following infection (Vallance and Collins, 1998).
CONCLUSION
Experimental studies in rodent model infections have greatly advanced our understanding of the various mucosal immune responses acting against GI nematode parasites. As important as these studies may be it is still worth bearing in mind that experimental studies are stereotypical; being generally conducted in inbred, and more recently, gene-KO and transgenic, animals under controlled environments and defined immunizing and challenge protocols. Thus, both the resulting infections, aspects of the immune resposes measured and the overall conditions of the animals may differ significantly from the situation under natural infections. However, such controlled conditions are necessary in order to achieve repeatability of results which would in turn allow analysis of the precise roles of individual factors and cell populations in the overall protective responses of the host against a particular nematode parasite or group of nematode parasites (Balic et al., 2000). We have based this review largely on such model system studies involving T. spiralis, T. muris, S. ratti, S. venezuelensis, N. brasiliensis and H. polygyrus whcih have provided most of our current understanding of mucosal immunity against GI nematodes. From these studies it is now evident that multiple effector mechanisms operate against GI nematodes and that the individual importance of each of the mechanism will vary depending on the host, species of parasites and the niche they occupy within the intestinal environment. This notwithstanding, two common features apparent from these studies are that protective immune responses are controlled by CD4+ Th2 lymphocyte responses and that resistance is impaired by CD4+ Th1 type responses. There is little doubt that anti-nematode immunity and the ability to expel nematodes from the gut depend on CD4+ T cells that can make Th2 cytokines and induce gut inflammatory responses, including mast or goblet cell responses and change in gut physiology all of which act in concert to create an environment hostile to worm survival and ultimately the dislogdement of adult worms from their intestinal niche within and expulsion from the host.
ACKNOWLEDGEMENTS
We thank Dr. Kenji Ishiwata for his help in preparing figure 2. DN Onah is a Postdoctoral Fellow sponsored by the Japan Society for the Promotion of Science (JSPS).